生物炭有机涂层对土壤养分的保持和肥力的促进作用

【参考文献】
Hagemann N, Joseph S, Schmidt H P, et al. Organic coating on biochar explains its nutrient retention and stimulation of soil fertility[J]. Nature communications, 2017, 8(1): 1089.
【摘要】
用生物炭(热解生物量)改良土壤是解决气候变化和土壤固碳能力退化、减少土壤温室气体排放和增加土壤养分保留的一种全球适用的方法。生物炭已经被证明能促进植物生长,特别是当与富含营养的有机物(如共堆肥生物炭)结合时。尽管对生物炭中养分储存的机制性理解尚不清楚,但生物炭促进植物生长的原因是养分的缓慢释放,在这里,我们通过高分辨率光谱(显微)和质谱分析鉴定了一种复合的、富营养的生物炭涂层,该涂层覆盖了生物炭颗粒的外表面和内(孔)表面。快速场循环核磁共振、电化学分析和气体吸附表明,该涂层增加了亲水性、氧化还原活性部分和额外的介孔性,增强了生物炭-水的相互作用,从而提高了养分的保留率。这意味着生物炭在土壤中的功能如先前所建议的是由有机涂层的形成决定的,而不是生物炭表面氧化。

【图表】
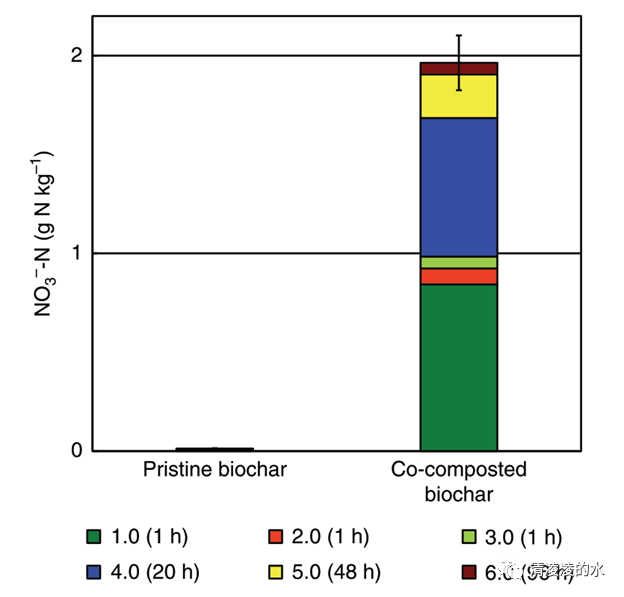
Fig. 1 Biochar nitrate content based on repeated extractions with 2M KCl. Each segment of the stacked bar represents one step of six consecutive extractions with KCl: the first, second and third extraction took 1 h each, the fourth took 20 h, the fifth 48 h, and the sixth took 95 h. Error bar represents 1 S.E. of the total extraction carried out in triplicates
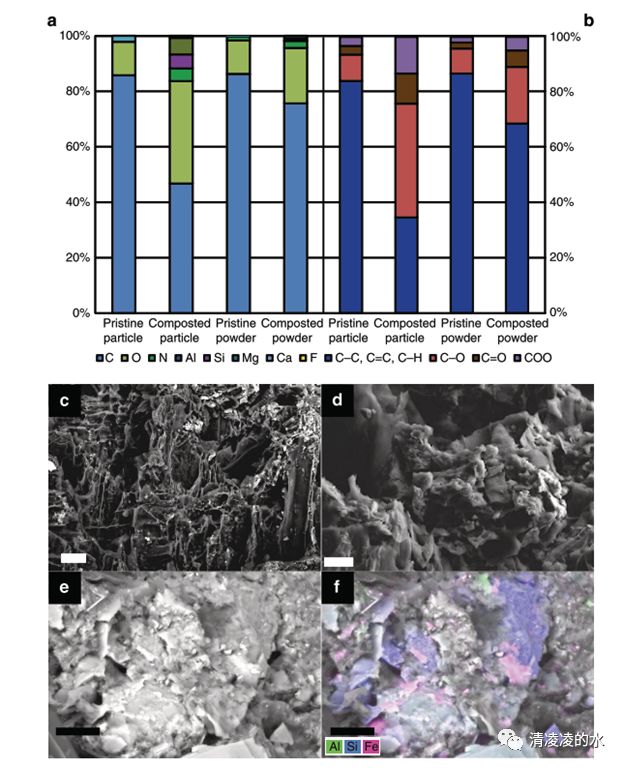
Fig. 2 Identification of biochar surface modifications induced by co-composting. a Elemental composition of the surface (particle) and the bulk (powder) biochar according to X-ray photoelectron spectroscopy (XPS). b Carbon-containing functional groups according to region scans (higher energy resolution) of the same samples. c–f Scanning electron micrographs of co-composted biochar with c a region with degraded, but still exposed original biochar surfaces showing typical porous structure (scale bar, 20 ?m); d a region of biochar surface partially covered with organic coating (scale bar, 10 ?m); e a region of biochar surface completely covered with organic coating (scale bar, 600 ?m). f map overlay of e showing the heterogeneous distribution of Al (green), Si (blue) and Fe (red) within the organic coating as quantified by energy-dispersive X-ray spectroscopy
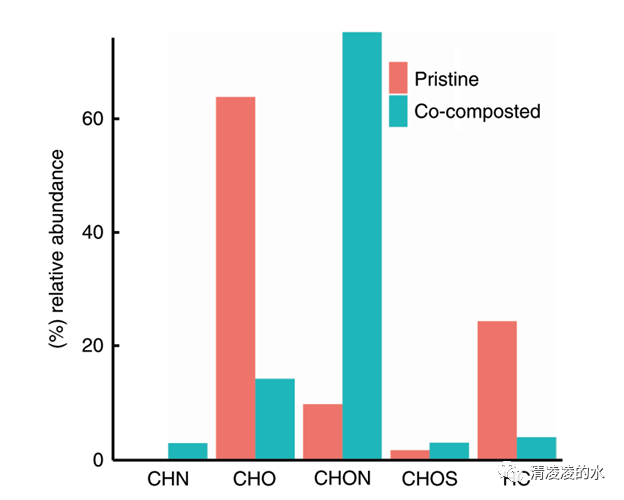
Fig. 3 In situ characterization of the organic coating by DAPPI FT-ICR MS. Relative abundance of C, H, O, N and S bearing compound classes on the surface of pristine and co-composted biochar according to desorption atmospheric pressure photoionization Fourier-transformed ion-cyclotron resonance mass spectrometry (DAPPI FT-ICR MS)

Fig. 4 Scanning transmission X-ray microscopy of co-composted biochar. a Average image on linear absorbance scale (optical density) calculated from a stack across the C1s absorption edge (278–330 eV) of an ultra-thin section of co-composted biochar. Scale bar, 500 nm. b C1s stack with overlay of regions of interest for downstream data evaluation: green— regions on or directly adjacent to the two biochar particles with relatively high N/C ratio (based on X-ray absorption ratio), red—manually defined region of the particle center, blue—all regions in the analyzed area with high N/C ratio, includes the green marked region. Same scale as a. c X-ray absorption spectra extracted from the regions defined in b with the respective colors, the blue spectrum again includes the green region. Spectra reveal a relative increase in absorption at the 1s-pi* transition (288.6 eV; R-COOH; CO62) from particle center (red) to particle associated high N/C (green) to all areas with high N/C (blue). In-depth analysis of this and two other regions of interest is displayed in Supplementary Fig. 3B, C. Comparison of with pristine biochar is displayed in Supplementary Fig. 3A

Fig. 5 Scanning transmission electron microscopy and electron energy loss spectroscopy of organic coating on co-composted biochar. Micrographs were obtained from different ultra-thin sections of the same biochar particle. a, b STEM HAADF micrograph and EELS spectra of an ultra-thin section of cocomposted biochar revealing an organic coating. Position 1: biochar with N and O below detection limit of EELS; 2: epoxy resin; 3: empty hole (resin did not penetrate this pore); 4: gold that was sputtered onto the biochar particle before embedding to identify the sample surface; 5: organic coating, porous appearance, contains N and O according to EELS spectra shown in b; 6 and 7: Location of EELS sum spectra shown in b. b EELS spectra revealing only minor differences in C speciation between biochar (red) and organic coating (blue), but considerably increased content of N in the coating. Letters indicate absorption edge (K shell) of C, N and O. c Closeup on a region of thick (~120 nm) organic coating, probably located inside a biochar pore (no gold coating detectable). d C-K near edge EELS spectrum of biochar matrix and coating as marked in c showing a peak shift of the C= C1s–π* transition (1) of aromatic carbon by ~0.5 eV (284.9–285.5 eV). C–C 2s-2pz σ* transition (2) was not altered (291.2 eV). e N-K near edge EELS spectrum of coating as marked in c showing (1) imine N 1s–π* transition at 399–400 eV; (2) amide N 1s–3p/π* transition at 401.3 eV; (3) nitro N 1s–π* transition at 403.6 eV; (4) corresponds to HC ≡ N* transition at 406.8 eV with σ* resonance position at 420.8 eV63. f Organic coating formed at a biochar surface that is rich in Ca hotspots of bright appearance in the HAADF micrograph. Coating is shearing off as an artefact of the mechanical force applied during sample preparation, most likely by the microtome, which indicates a rather plastic nature of the coating compared to the biochar that broke (lower part of the micrograph). Thickness of coating varies between ~20 and 50 nm and the presence of gold indicates a semi-exposed position in the original biochar particle. g EELS spectra from regions indicated in f showing the peak shift of the C =C1s–π* transition and the presence of Ca in the region of the bright spot in the outermost region of the biochar
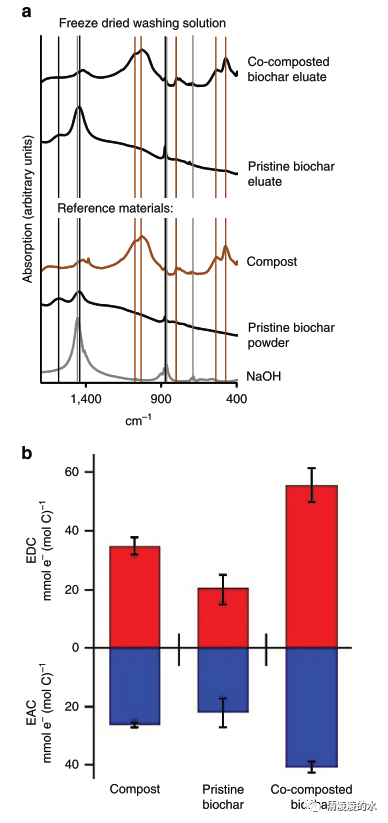
Fig. 6 Ex situ analysis of the organic coating extracted from the biochar with 0.05M NaOH. a Fourier-transformed infrared (FTIR) spectra of freeze-dried eluates of pristine and co-composted biochar. FTIR spectra of pure compost, pristine biochar and NaOH were measured as a reference. b Electron accepting (blue) and electron donating (red) capacity (EAC and EDC) of biochar eluates normalized to their carbon content. Error bars represent one standard deviation of at least five replicates
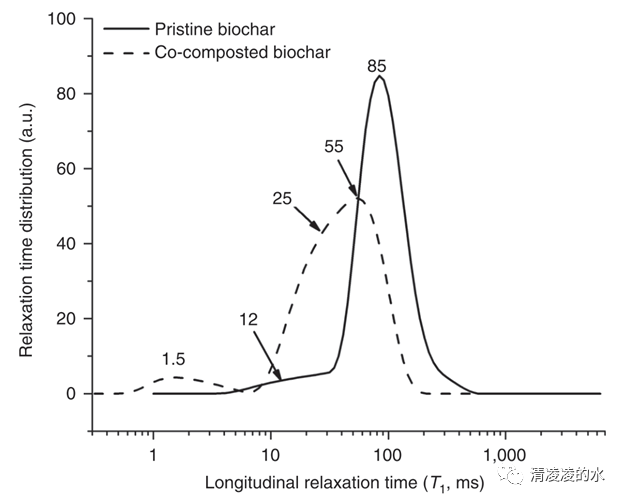
Fig. 7 Analysis of biochar surface hydrophilicity by fast field cycling nuclear magnetic resonance relaxometry: Relaxograms, i.e., distribution of relaxation times, of pristine and co-composted biochar. The shorter the T1 value, the better water molecules are anchored to the biochar surface. Conversely, the longer the longitudinal relaxation time, the weaker are the interactions between water and the surface of the biochar system
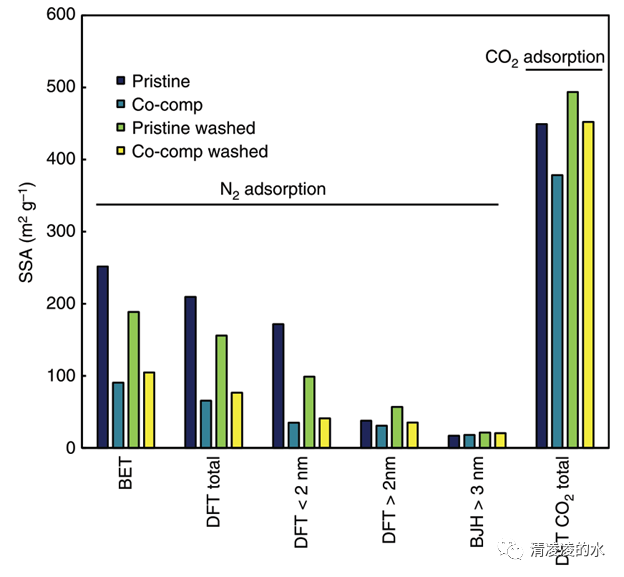
Fig. 8 Specific surface area (SSA) according to N2 and CO2 gas adsorption. Total SSA according to the Brunauer, Emmett and Teller method, total SSA according to the quenched-solid density functional theory (QSDFT) method, SSA due to micropores according to the QSDFT method, SSA for mesopores up to 34 nm according to the QSDFT method, SSA for pores bigger than 3 nm according to the Barrett, Joyner and Halenda method and SSA of micropores with CO2 adsorption and applying the non local density function theory method of pristine and co-composted biochar, pristine biochar after washing and co-composted biochar after washing

